The inhibition of nociceptive pathways reduces the sensory and emotional aspects of pain. Understanding these pathways, allows the selection of appropriate agents and the ethical treatment of a variety of species in veterinary practice. This article will concentrate on the pain pathways, the pharmacology of opioids and their role in administration of analgesia. As veterinary literature can be limited this analysis will use a combination of human and veterinary studies to provide a more comprehensive understanding.
Pain pathways
Pain is an unpleasant experience providing sensory and emotional components to actual or potential tissue damage (McKune et al, 2015).
Physiologic pain is detected and interpreted by a nociceptive sensory system, responding to pain through transduction, transmission, modulation, and perception (McKune et al, 2015).
Transduction and transmission
Peripheral nociceptors detect noxious (thermal, mechanical, and chemical) stimuli and an action potential is transmitted by two types of primary afferent neuron: C-fibres and A-δ fibres (Dubin and Patapoutian, 2010). A-δ fibres are lightly myelinated fibres with a diameter of 2–5 µm (Figure 1), and have a low threshold for noxious stimuli (thermal and mechanical), providing rapid delivery of action potentials in response to acute pain. C-fibres are unmyelinated and have a diameter of <2 µm (Figure 1), and provide slow delivery of action potentials. C-fibres have a high threshold and are polymodal; therefore, they respond to chemical, mechanical, and thermal noxious stimulation (Reddi et al, 2013).
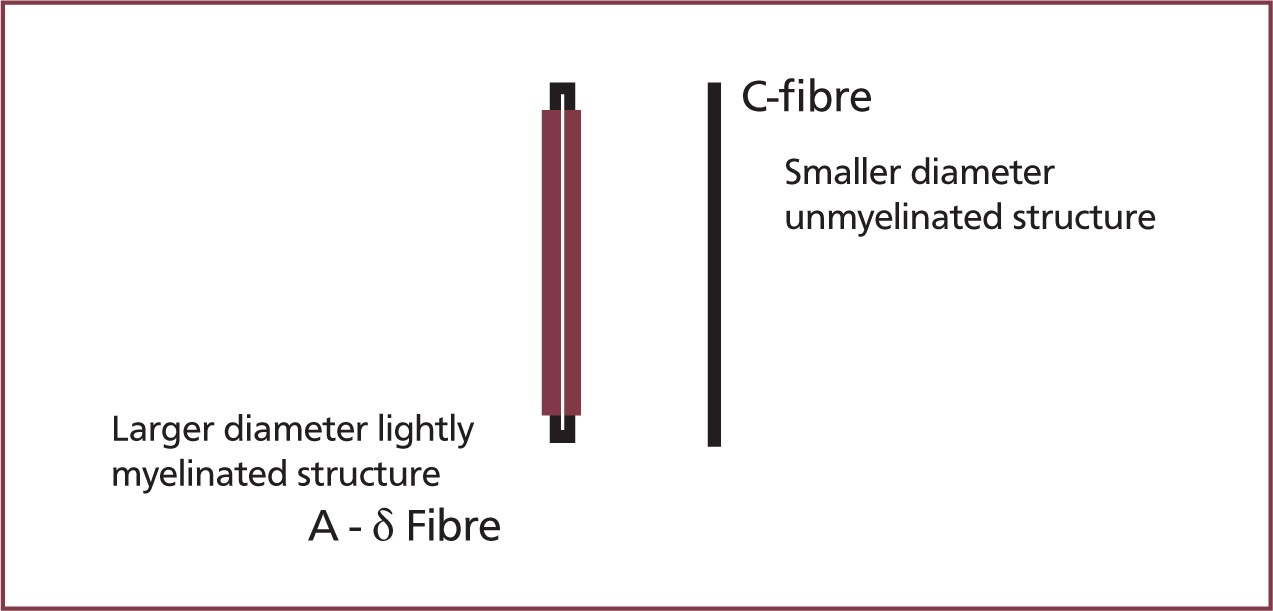
Sensitisation can be influenced by inflammatory mediators (bradykinin, serotonin, prostaglandins, cytokines, and H+) released from damaged tissue, affecting the nociceptors directly, and lowering the threshold for activation in a process called primary sensitisation (Reddi et al, 2013).
Modulation
Action potentials are carried to synapses at the dorsal horn of the spinal cord — C fibres terminate within lamina II and A-δ fibres terminate within lamina I and V of the spinal cord. This triggers the release of neurotransmitters: glutamate activates α-amino-3-hydroxy-5-methyl-4-isoxazolepropionic acid (AMPA) receptors; substance P activates neurokinin 1 (NK1) receptors; and calcitonin gene-related peptide (CGRP) activates CGRP receptors (McKune et al, 2015). The activation of receptors causes an efflux of ions and subsequent depolarisation of the second-order neurons.
N-methyl-D-aspartate (NMDA) receptors on the second-order neurons are typically inactive; however, in times of prolonged depolarisation, for example where there is chronic pain, the receptor can become active. This causes a lowered activation threshold and the release of nitric oxide, increasing release of neurotransmitters.
Perception
Second-order neurons synapse with third-order neurons and action potentials are projected to the cortex, concluding the ascending pathway and the brain's perception of the stimuli (Figure 2).
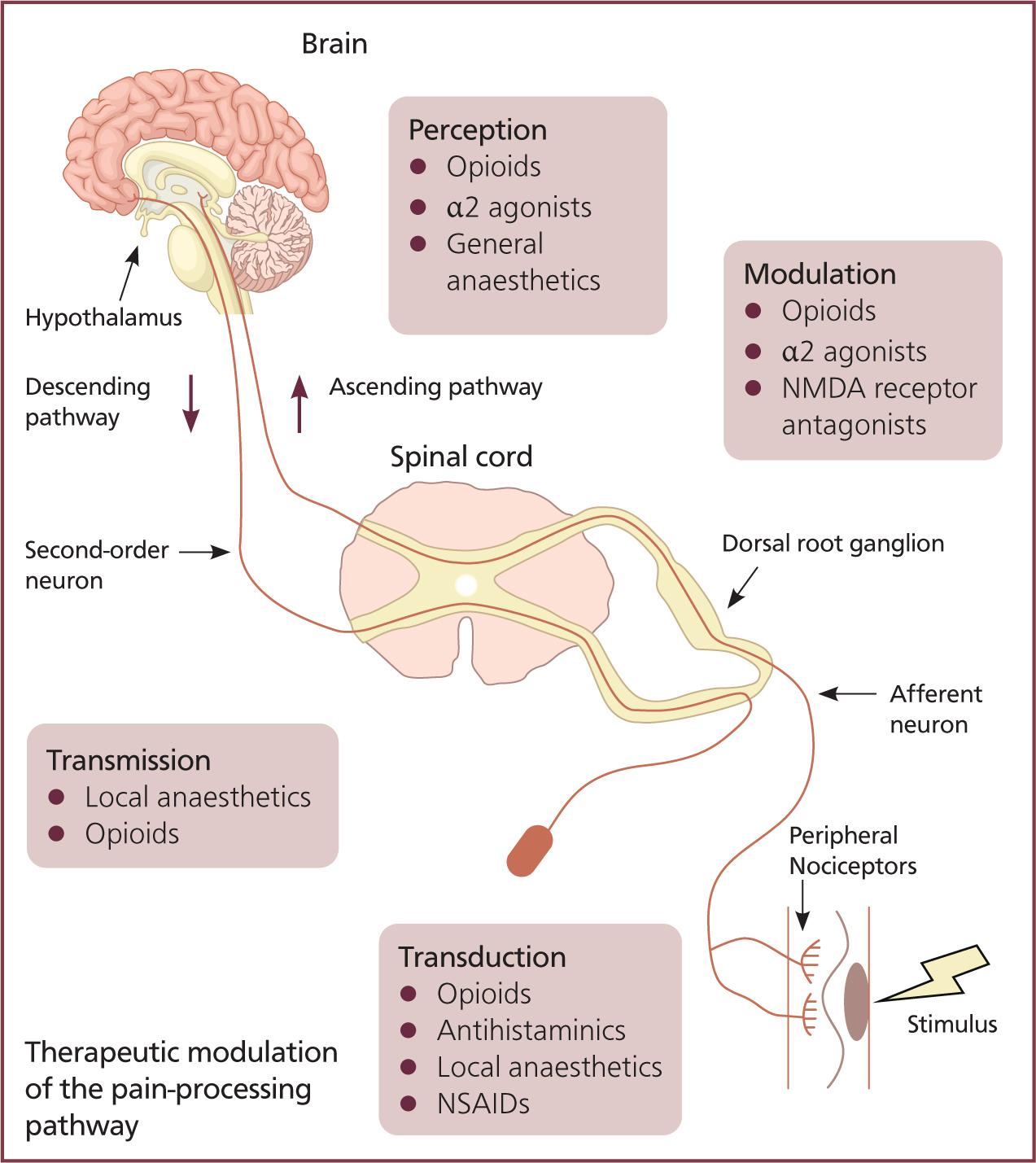
Descending pathway
The descending pain modulation pathway begins at the periaqueductal grey matters in the midbrain — the stimulating action potential passes through neurons to the nucleus raphe magnus. An action potential is then relayed to the dorsal horn via noradrenergic and serotonergic neurons. The release of serotonin and noradrenalin inhibits first-order neurons and causes interneurons to release enkephalin (a natural opioid). Enkephalin inhibits the release of first-order presynaptic neurotransmitters and the depolarisation of second-order neurons; reducing the delivery of nociceptive signals (Dubin and Patapoutian, 2010).
Opioids
Opioids are natural and synthetic substances that have an affinity for opioid receptors (Boothe, 2012).
Receptors include:
- Mu opioid peptide (MOP)
- Kappa opioid peptide (KOP)
- Delta opioid peptide (DOP)
- Nociceptin orphanin FQ peptide (NOP).
Different receptor activity is linked to varying clinical usage.
Mu opioid peptide mechanism of action
MOPs are coupled to inhibitory G-proteins; activation leads to calcium channels closing on the pre-synaptic neuron. This results in hyperpolarisation by potassium efflux at the post-synaptic neuron and inhibition of adenylyl cyclase, reducing cyclic adenosine monophosphate (cAMP). This series of events inhibits neurotransmitter release such as glutamate, substance P, and CGRP at pre-synaptic afferent neurons and consequently there is a reduction in nociceptive impulses (Peck and Hill, 2014a).
Side effects of opioids
In addition to analgesia MOP agonists can have profound effects on other body systems, which can be detrimental dependent on dose and morbidity of the patient (Table 1). Opioids can cause sedation, dysphoria, euphoria, and depression of cardiovascular, respiratory, endocrine, and gastrointestinal (GI) systems.
Table 1. Side effects of opioids
Body system | Effect | Reason |
---|---|---|
Cardiovascular | Bradycardia | Reduced sympathetic drive and direct effect on the sinoatrial node. Effect on blood pressure can be more pronounced with selective opioids that cause histamine release (Trivedi et al, 2007) |
Respiratory | Inhibited | Reduction in responsiveness of the brainstem to carbon dioxide |
Endocrine | Varied | Inhibition of adrenocorticotropic hormone (ACTH), prolactin and gonadotrophic hormone, and increased production of antidiuretic hormone (ADH) (Trivedi et al, 2007) |
Gastrointestinal | Ileus | Increase in smooth muscle tone, reduced motility resulting in delayed absoption and constipation, nausea, and vomiting |
Reproductive | Transfer of opioids through the umbilical cord | Systemic administration can pass to a fetus via the umbilical cord, this is reduced in less lipophilic molecules |
Distribution of opioids
The primary effect site for opioids is the central nervous system (CNS), but they also diffuse rapidly to vessel rich tissues such as the brain, liver and kidney to equilibrate (KuKanich and Wiese, 2015).
A loss of drug effect initially occurs as a result of metabolism and distribution to poorer perfused tissues, causing the opioid to move from the CNS to maintain plasma equilibrium. Repeat opioid administration can result in tolerance, causing a right shift in the dose response curve (Figure 3).
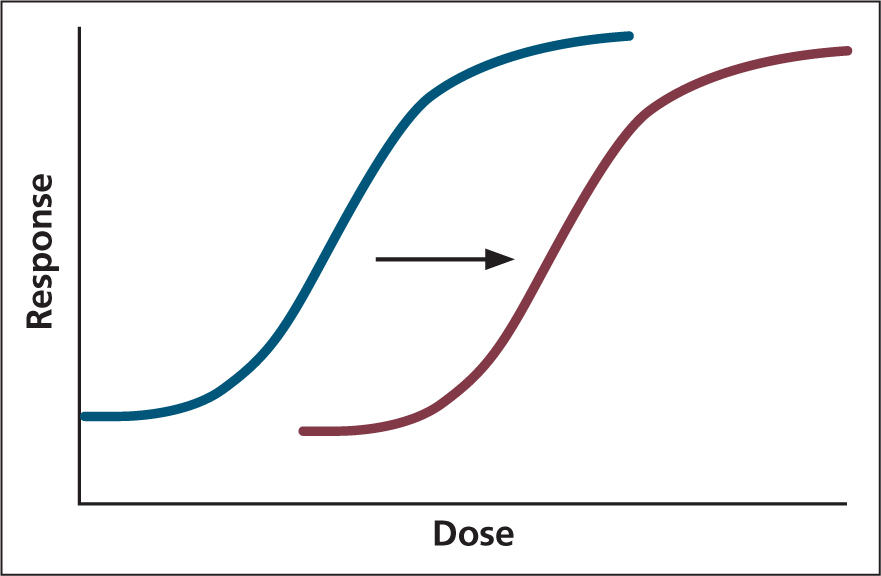
Tachyphylaxis is the term used for a decreased response to a drug.
Tolerance is long-term reduction in response to the same dose (Maxwell, 2012). However, although tolerance results in an increased dose of opioid required to provide the same level of analgesia, the sensitivity to side effects is relatively unchanged. Therefore, patients with chronic pain, receiving opioids as part of a pain management protocol prior to surgery, may exhibit severe respiratory depression, nausea, and GI dysfunction in the postoperative period (Dumas and Pollack, 2008; White et al, 2017).
Full and partial agonists
Particular pharmacodynamics contribute to the effectiveness of a MOP agonist; particularly whether a full agonist or partial agonist is administered.
Partial agonists have a high affinity for the MOP and can be highly potent. However, a ‘ceiling’ effect limits the analgesia they provide, whereby at a certain point a further increase in dose will not provide further population response (Peck and Hill, 2014a). This is something to carefully consider when administering these drugs (e.g. buprenorphine), as these properties will substantially limit analgesia options until drug clearance. Therefore, it is often more appropriate to use low doses of a full agonist that can be titrated to effect.
Full agonists provide a dose-dependent response: high doses will provide more side effects as previously discussed, therefore, a multimodal approach should be utilised. When examining the logarithmic dose response curve for full agonists and partial agonists, the ED50 (the concentration of a drug required to elicit a response in 50% of the population administered) can be lower in some partial agonists; however, unlike full agonists they will never reach 100% population response (Lambert, 2004).
As a result of partial agonists' higher affinity for the MOP, they can act as competitive antagonists for full MOP agonists. This property also limits analgesic techniques once a partial agonist has been administered (Peck and Hill, 2014a).
Fentanyl
Fentanyl is a synthetic phenylpyperidine opioid, a full MOP agonist and is 100 times more potent that morphine (Figure 4). Fentanyl has a high lipid solubility — it is nearly 600 times more lipid soluble than morphine — causing rapid onset of action and in low doses, a short duration of action (20 minutes) because of its high volume of distribution (10 litres/kg) (Boothe, 2012; Peck and Hill, 2014b). When given in high doses or by infusion over time, tissues become saturated and elimination time can be significantly extended with half-life of 3 hours (Boothe, 2012).
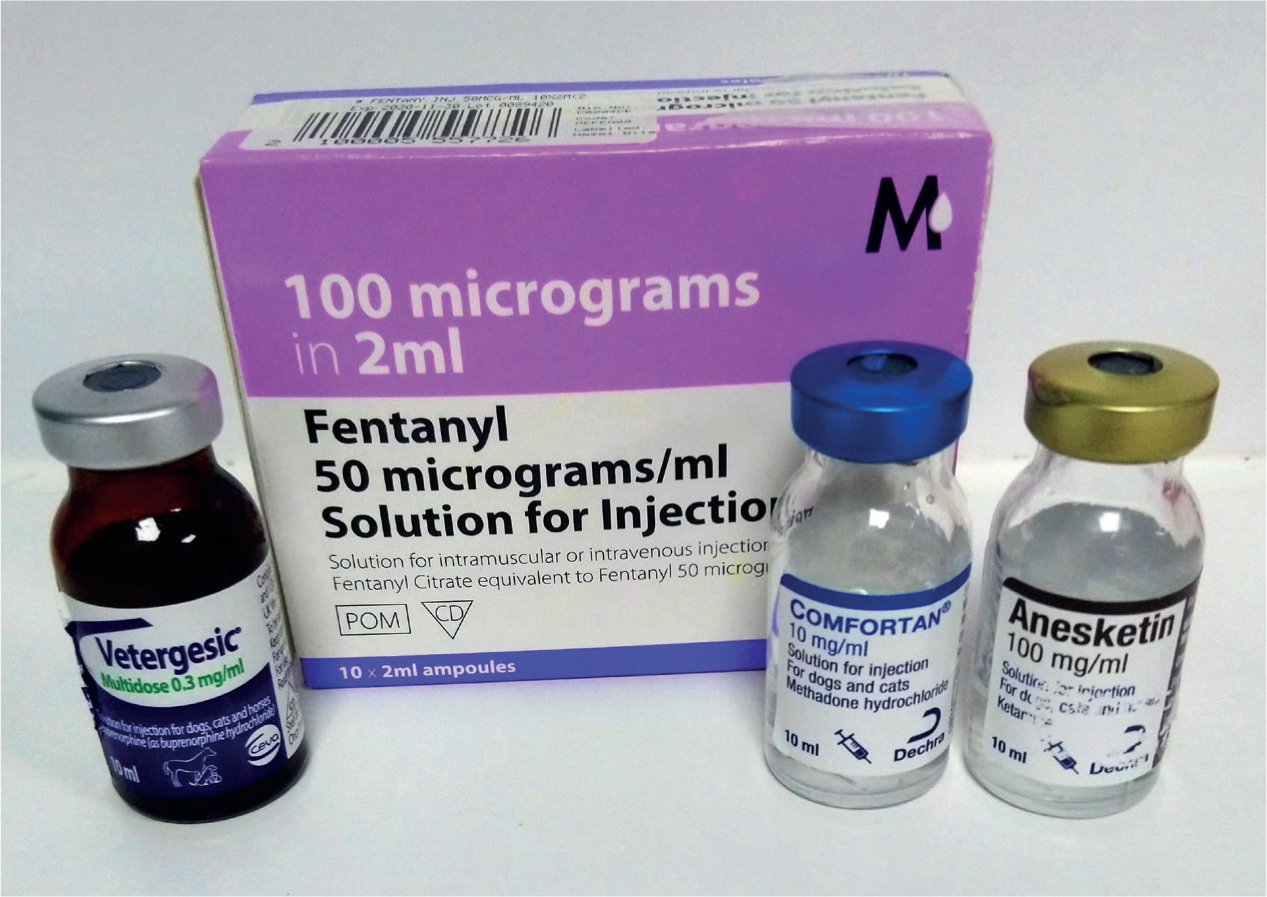
Fentanyl has a high affinity for MOP receptors and Robinson et al (1999) showed fentanyl to provide superior analgesia to morphine during major orthopaedic surgery. It causes minimal cardiovascular suppression when compared with other opioids, although respiratory depression with profound sedation is commonly seen (Boothe, 2012).
Administration is intravenously (IV), intramuscularly (IM), by mouth (PO), intranasally and transdermally.
Intravenous route
- Most common route
- Short duration of action in clinical doses
- Bolus: 1–5 μg/kg in dogs and cats
- Follow bolus with constant rate infusion (CRI): 2.5–10 μg/kg/hour (titrated to effect).
Some sources will provide larger dose ranges such as 5–40 μg/kg/hour (Kerr, 2016); however, care should be taken particularly if unfamiliar with the effects of fentanyl. It could also be argued, although beyond the scope of this article, that a more multimodal approach is more appropriate if the lower dose range is ineffective, as it will become clear from the discussion of side effects.
Transdermal patches
- Transdermal patches are useful for longer periods of analgesia (24–72 hours)
- Particularly useful for patients that will not tolerate IV cannulation
- Takes considerable time to reach peak plasma concentrations:
- Dogs — 24 hours
- Cats—8–73 hours (highly variable)
Transdermal solutions
- For dogs — available lasting up to 96 hours
- Should be hospitalised and monitored for first 48 hours before discharge, because of the side effect of hypothermia
- Caution — risk to humans by accidental exposure or opioid abuse (Kyles et al, 1996; Egger et al, 2003Ramsey, 2014).
Metabolism
Fentanyl undergoes phase I enzyme metabolism via cytochrome P450 (CYP) mechanisms in the liver, and clearance is at 20–77.9 ml/minute/kg and is influenced by hepatic blood flow (Riviere and Qiao, 1999). The exact mechanism in dogs is unknown, but in humans and rats, enzyme metabolism is by isozyme CYP3A4 and CYP3A1/2 respectively (Feierman, 1996; Labroo et al, 1997). This mechanism of dealkylation produces the metabolite norfentanyl, which is excreted by both urinary and biliary routes.
Cytochrome P450 metabolism
CYP metabolism varies between breeds and species, with Greyhounds exhibiting deficiency in CYP2B11 responsible for propofol metabolism, resulting in delayed recovery (Zoran et al, 1993). Although this has not been an identified effect on opioid metabolism in dog breeds, it supports administering opioids to effect and with individually assessed pain management plans. Another factor that can affect the CYP pathway is interactions with CYP inhibitory or inducing agents. Ketaconazole, a CYP inhibitor, was shown to decrease metabolism in other drugs, but only demonstrated a small change in fentanyl — elimination of fentanyl was not significantly altered by co-administration of ketoconazole with the mean residence time increasing from 3.90 to 6.35 hours (KuKanich and Hubin, 2010).
Fentanyl is a diverse and effective drug for the provision of analgesia in clinical practice. It is commonly used as a bolus in addition to other opioids for short acting perioperative analgesia, infusions as a titrated adjunct analgesic, and as part of a postoperative pain management plan, whether by CRI or transdermal administration. This opioid is highly potent, provides short-to long-term action and dose-dependent analgesia. Fentanyl is superior in analgesic efficacy, potency and versatility when compared with other opioids.
Methadone
Methadone is a synthetic diphenylpropylamine, providing similar analgesic effect to morphine (Figure 4). This full MOP agonist consists of a racemic mixture of two stereoisomers: L-methadone and D-methadone (KuKanich and Wiese, 2015). D-methadone has NMDA antagonist properties, and L-methadone has 10–50 times greater affinity for MOP receptors than D-methadone (Boothe, 2012).
Methadone is licensed in the UK for use in dogs at a dose range of 0.5–1.0 mg/kg by intravenous, intramuscular and subcutaneous route, and in cats at a dose range of 0.3–0.6 mg/kg by intramuscular injection (Veterinary Medicines Directorate, 2018). This licensing indicates that methadone should be used in place of other alternatives such as morphine, unless the situation dictates an alternative under the cascade. Clinical usage of methadone is typically at lower doses, and with intravenous and subcutaneous routes being used in cats (Ramsey, 2014).
Administration of methadone
- Administration is by intravenous and intramuscular routes—half-life of 4 hours with high volume of distribution of 3.4 litres/kg (KuKanich et al, 2005a)
- Because of its long half-life and accumulation properties, this drug is not appropriate for CRI administration
- Epidural administration provides 8 hours of analgesia duration
- Subcutaneous administration can provide greatly prolonged half-life of 10.7 hours +/- 4.3 hours (Ingvast-Larsson et al, 2010)
- Methadone is not administered orally because of its low bioavailability resulting from first pass metabolism (KuKanich et al, 2005a).
Methadone is used for the provision of pre-emptive analgesia, intraoperative (at lower and carefully titrated doses) and postoperative pain management. With repeat dosing, an accumulation can occur, resulting in a steady increase in plasma concentration over time; this can result in intermittent toxicity or suboptimal analgesia between administration. Therefore, it is advisable to regularly reassess the patient and often reduce subsequent doses. Dose reduction can be more appropriate than extending dosing intervals, as it prevents these large peaks and troughs in plasma concentration.
Side effects of methadone
- Occasional transient excitement seen after intravenous administration
- Respiratory depression
- Constriction of GI sphincters
- Pupillary dilation in cats, that can temporarily impair vision.
- On intravenous administration, methadone is not associated with histamine release and does not generally induce emesis when given pre-emptively; this is a clear advantage over morphine.
Distribution
As previously discussed, lipophilic drugs redistribute to tissues and it is this movement from the central compartment that can be responsible for initial recovery. It has been theorised that all lipophilic drugs will have reduced distribution in lean dogs (Sams et al, 1985); however, greyhounds showed a greater volume of distribution (7.79 ± 1.87 litres/kg) than beagles (3.5 ± 1.1 litres/kg) (KuKanich and Borum, 2008), supporting an individual approach to pain management to achieve optimal plasma concentrations.
Metabolism
Metabolism of methadone is thought to be primarily phase I in the liver, with human studies showing CYP system (CYP3A4) being responsible for the N-demethylation to an inactive metabolite (2-ethylidene-1,5-dimethyl-3,3-diphenylpyrrolidine) (Ferrari et al, 2004). However, in the dog this metabolite has not been isolated, and unchanged methadone was found in urine (3%) and faeces (<1%), with multiple unidentified metabolites (Garrett et al, 1985). This indicates an unidentified CYP pathway is responsible for metabolism of methadone in canines. As previously discussed, this pathway can be influenced by breed and by interactions with CYP inhibitory or inducing agents. The administration of ketoconazole (CYP inhibitor) in canines produced a peak concentration 30 times greater than if the ketoconazole had not been administered, after oral administration of methadone (KuKanich et al, 2011); this has clinical implications when considering dose and administration intervals.
Morphine
Morphine is the opioid that all others are compared with and has a relative potency of 1. It is isolated from the plant Papaver somniferum (commonly called the opium poppy), and is a natural opioid with a high affinity for the MOP receptor and low affinity for the KOP and DOP (Monteiro et al, 2009). Morphine provides efficacious analgesic action but as it is unlicensed in the UK for use in companion animals, it is rarely used in place of methadone.
Administration of morphine
Recommended doses are provided in Table 2.
- Poor lipid solubility makes morphine advantageous for epidural administration because of the slower absorption by the CNS allowing greater distribution (Steagall et al, 2017)
- Bioavailability by oral administration is very poor (5–20%) as a result of first pass metabolism and metabolism by intestinal mucosa (Aragon et al, 2009).
Table 2. Morphine recommended dosages
Recommended dosages | |||
---|---|---|---|
IV, IM, SC | CRI | Epidural | |
Dog | 0.5 mg/kg (half-life 60 mins) | 0.15–0.2 mg/kg/hour (titrated to efect) | 0.1 mg/kg (diluted) Duration: 18–24 hours |
Cat | 0.1–0.4 mg/kg (half-life 75 minutes) | Not well studied in cats | 0.1 mg/kg (diluted) Duration: 18–24 hours |
IV = intravenous, IM = intramuscular, SC = subcuraneous, CRI = contast rate infusion
Taylor et al, 2001; KuKanich et al, 2005bAlthough methadone would be used in place of morphine in most cases, because of its short half-life and epidural administration, morphine's properties make it a more desirable drug for infusions, and it can be used under the cascade.
Side effects of morphine
Morphine has similar effects of sedation, euphoria, dysphoria, respiratory depression, bradycardia, ileus, and urinary retention as other opioids.
The concern with intravenous administration of morphine is the possibility of transient excitement and histamine release. Histamine release is not typically seen in other opioid administration and can cause constriction of conducting airway and hypotension, severely impacting on tissue perfusion, particularly in patients with pulmonary pathology (Peck and Hill, 2014b). Administration of morphine pre-emptively is associated with nausea and vomiting as a result of stimulation of the chemoreceptor trigger zone.
Metabolism
Metabolism of morphine occurs in the liver, kidney, and brain (Yamada et al, 2003; Peck and Hill, 2014b). In dogs only 50% of metabolism is hepatic compared with near complete hepatic metabolism in other opioids (Jacqz et al, 1986). In cats the clearance of morphine is 24 ml/kg/minute (Taylor et al, 2001); approximately half the rate seen in the dog (Dohoo et al, 1994). Phase I metabolism through CYP as seen in other opioids does not occur to any great extent in morphine (Holmquist, 2009). Phase II metabolism in the cat occurs rapidly by sulphation compared with glucuronidation in the dog (Taylor et al, 2001).
The major metabolite (70%) produced in dogs and humans is morphine 3-glucuronide (M3G). It is an inactive analgesic with MOP antagonist properties (Peck and Hill, 2014b; KuKanich and Wiese, 2015). Morphine 6-glucuronide (M6G), an active metabolite produced in far smaller quantities, has an efficacious analgesic effect and is 13 times more potent than morphine. Both metabolites are excreted in the urine; therefore, accumulation can occur in cases of renal insufficiency (Mazoit et al, 2007).
Epidurals
Epidural administration close to the site of action allows more profound analgesia at lower dose rates resulting in fewer and less severe adverse side effects (Torske and Dyson, 2000). When a local anaesthetic such as bupivacaine is added, the level of analgesia and the duration of action can be extended (Troncy et al, 2002).
For Caesarean sections, this understanding can be applied, as the provision of opioids reduces inhalant agents, provides analgesia, and therefore supports haemodynamic stability. Romagnoli et al (2019) showed that pre-emptive epidural administration of methadone (0.1 mg/kg) provided a comparable level of analgesia to intramuscular administration (0.3 mg/kg), but with far lower umbilical cord concentrations.
Conclusion
Pharmacology of opioids is vast and not completely understood; however, knowledge of mechanisms of action and elimination can greatly improve a patient's analgesia treatment plan. The selection of the most appropriate opioid and administration via the most advantageous route optimises provision of analgesia and minimises negative side effects.
This application of basic pharmacology should be applied to all cases requiring analgesia from those in first opinion to more complex referral cases.
KEY POINTS
- An awareness of side effects of opioids should encourage careful dosing and utilisation of multimodal techniques.
- Half-life and metabolism of a particular opioid should direct dose and dosing intervals, avoiding a protocol for everyone approach.
- Dosing of opioids should be titrated to effect as much as possible, regularly assessing the patient as an individual, to avoid undesirable side effects.
- Poor analgesic response or significant side effects should not be ignored, particularly if standardised protocols for all patients are being implemented. Always reassess the plan and be prepared to alter the dose +/- add an adjunct analgesic agent.
- When considering the route of administration, factors that should be considered are: minimising pain to the patient (IV administration where possible), skill of staff (can epidurals be considered?) and the distribution characteristics of the opioid.