As veterinary medicine is continuously evolving, so are the expectations for the level of patient care. In the veterinary emergency and critical care (ECC) setting, patient care checklists can be utilised to optimise patient care quality and standards. Checklists create a step-by-step process of evidence-based interventions and procedures that help prevent medical oversights (Berenholtz et al, 2002; Fulbrook and Mooney, 2003).
Kirby's Rule of 20 is a checklist created by Rebecca Kirby, DVM, DACVIM, DACVECC that includes 20 patient parameters that should be evaluated daily in critically ill patients. Kirby's Rule of 20 was created as a reminder for veterinarians and veterinary nurses to examine the status of critically ill patients, including organ systems involved, clinical parameters, diagnostic parameters, and treatment goals in order to optimise patient survival (Kirby, 2017). Following the Kirby's Rule of 20 checklist allows veterinary nurses to assess the overall clinical picture of a patient (taking a holistic approach), implement critical thinking skills, elevate the quality of patient care, set standards for patient care, and decrease morbidity and mortality which results in improved patient outcomes (Berenholtz et al, 2002; Fulbrook and Mooney, 2003).
The patient parameters in the Kirby's Rule of 20 checklist are:
- Fluid balance
- Albumin and oncotic pull
- Electrolyte and acid–base
- Mentation
- Heart rate, rhythm and contractility
- Blood pressure
- Body temperature
- Oxygenation and ventilation
- Red blood cells (RBCs) and haemoglobin
- Coagulation cascade
- Renal function
- Gastrointestinal (GI) motility and integrity
- Nutrition
- Glucose
- Immune status and antibiotics
- Wound healing and bandages
- Drug dosage and metabolism
- Pain control
- Nursing care
- Tender loving care
To allow enough detail for each parameter, part 1 will focus on the physiology, clinical application, and monitoring of patient parameters 1–5.
Fluid balance
Fluid balance refers to a state of total body water (TBW) homeostasis (Kirby and Rudloff, 2017). Physiologically speaking, the body's fluid compartments are divided between intracellular (ICF) and extracellular (ECF) (Silverstein and Santoro-Beer, 2015). The ICF comprises fluid within the cell while the ECF comprises fluid outside the cell in the intravascular and interstitial. The sum of each fluid compartment equates TBW (Figure 1).
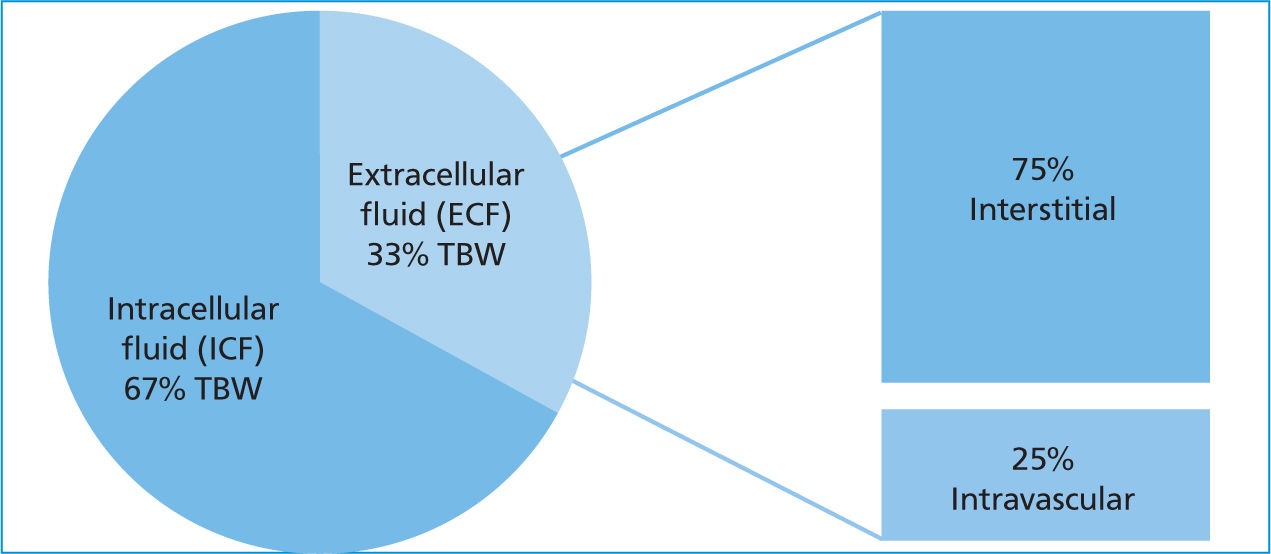
Fluid imbalances occur from disease conditions that alter a patient's ability to adequately compensate and restore its own fluid requirements, thus requiring fluid therapy intervention. Definitions that are part of fluid balance include hypovolaemia and dehydration. Hypovolaemia is loss of intravascular fluid volume (i.e. hemorrhage, vomiting/diarrhoea, urinary loss). Dehydration is loss of interstitial and intracellular fluid volume (i.e. decreased water intake in relation to water lost) (Kirby and Rudloff, 2017).
Intravenous fluids are used in the critically ill to maintain intravascular volume and adequate perfusion (Liss and Norkus, 2019). The mainstay fluid type for treating critical patients are isotonic crystalloids as they have the most similar composition to the patient's ECF compartment. Crystalloids are a water-based solution composed of osmotically active small molecules that are permeable to capillaries (Silverstein and Santoro-Beer, 2015). It is important to remember that a patient's fluid therapy plan must be tailored to every patient and requires reassessment and recalculation of fluid needs.
When developing a fluid therapy plan, factors such as the patient's level of disease, life stage, hydration status, and ongoing losses must be taken into consideration (Scalf, 2014). To begin, the maintenance fluid requirement refers to the body's fluid needs in order to maintain homeostasis and is calculated as 40–60 ml/kg/day (Silverstein and Santoro-Beer, 2015). Disease states that require more aggressive fluid therapy include hypovolaemia, renal disease, vasodilatory states, and certain toxicities. Disease states that require more conservative fluid therapy include cardiovascular compromise and electrolyte derangements. Dehydrated patients will require additional fluid support on top of maintenance fluid requirements. Replacement for dehydration is calculated using the dehydration deficit: % dehydrated x kg x 1000 = volume to be replaced in milliliter (ml) (Silverstein and Santoro-Beer, 2015). The dehydration deficit should be replaced over 4–24 hours and is an additional rate to the hourly maintenance requirement (Kirby and Rudloff, 2017). Patients with ongoing losses (i.e. hemorrhage, profound vomiting/diarrhea, excessive urinary loss) will also need to have that volume quantified and replaced every 4–24 hours (Liss and Norkus, 2019).
To ensure adequate monitoring of fluid therapy, a variety of parameters are assessed, including haemodynamic parameters (i.e. heart rate, respiratory rate, blood pressure), ongoing losses, hydration status, serial bodyweight, and lab values (packed cell volume (PCV), total protein (TP), blood urea nitrogen (BUN), creatinine) (Darbo and Page, 2017). Patients receiving fluid therapy should also be monitored for signs of fluid overload (overhydration), which is a state of excess fluid content in the interstitial and intracellular spaces (Kirby and Rudloff, 2017). Clinical signs of fluid overload include serous nasal discharge, chemosis, subcutaneous oedema, ascites, increased respiratory rate, coughing, and restlessness (Donohoe, 2014).
Albumin/oncotic pull
Albumin and oncotic pull are closely related to fluid balance, specifically when looking at the relationship of Starling's Forces. Starling's Forces refers to the forces that dictate water movement within the ECF fluid compartment (Kirby and Rudloff, 2017). Fluid movement occurs across capillary membranes based on hydrostatic pressure (the force that pushes water out of the intravascular space) and oncotic pressure (the force that holds water within the intravascular space) (Silverstein and Santoro-Beer, 2015).
Albumin is the predominant protein within the intravascular space and is responsible for maintaining vascular integrity and colloid oncotic pressure (COP). Decreased oncotic pressure results in fluid movement into the interstitial space (Odunayo, 2017). When critically ill patients have a lowered albumin level (i.e. hypoalbuminaemia) as a consequence of their underlying disease, the protein can no longer retain fluids within the vascular space and the result is increased intravascular permeability leading to thirdspacing and oedema (Liss and Norkus, 2019).
Hypoalbuminaemia is the result of pathological events and should be anticipated as a complication of any critical illness (Odunayo, 2017). Hypoalbuminaemia can result from loss through bleeding, loss through wound seepage, dilution from intravenous fluids, reduced synthesis of albumin, malnutrition, renal disease (i.e. protein-losing nephropathy, proteinuria), and gastrointestinal (GI) disease (protein-losing enteropathy) (Odunayo, 2017).
Monitoring albumin and COP involves assessment of patient parameters (i.e. heart rate, respiratory rate, blood pressure), cavitary effusions (i.e. pleural effusion, peritoneal effusion), tissue oedema, and laboratory values (i.e. PCV, TP) (Odunayo, 2017). Hypoalbuminaemic patients can be supported by administering colloids to increase and maintain COP (Odunayo, 2017). Natural colloids are derived from blood products and contain naturally occurring albumin (i.e. fresh frozen plasma, frozen plasma, whole blood, human serum albumin) (Odunayo, 2017). Synthetic colloid solutions contain large molecules suspended in crystalloid solutions that help maintain intravascular volume because they do not as readily cross the capillary walls (i.e. hydroxyethyl starches, dextrans) (Scalf, 2014).
Electrolytes and acid–base
Electrolytes are essential, electrically charged particles within the ICF and ECF compartments. Sodium, potassium, chloride, phosphorus, calcium, and magnesium all play a vital role in maintaining normal cellular functions (Scalf, 2014).
Sodium is the body's primary extracellular cation, and is responsible for maintaining the ECF balance in the body, which helps maintain effective circulatory volume (Randels-Thorp and Liss, 2016). Sodium levels are maintained within narrow limits by balancing water intake and water loss (Barton and Kirby, 2017).
Potassium is the body's primary intracellular cation, and it is responsible for maintaining the resting potential of muscle cells (i.e. cardiac, skeletal) (Randels-Thorp and Liss, 2016). Total body potassium concentration is tightly regulated by the kidneys matching excretion with intake (Barton and Kirby, 2017).
Chloride is the body's primary extracellular anion, and it is responsible for regulating osmotic pressure differences between fluid compartments and influences acid–base balance (Randels-Thorp and Liss, 2016). Chloride levels are maintained through secretion and reabsorption through the kidneys (Barton and Kirby, 2017).
Phosphorus is the body's major intracellular anion, and it is responsible for the structure of cells (i.e. phospholipid bilayer of cell walls), adenosine triphosphate (ATP) synthesis, glucose transport, lactate production, and cellular metabolic processes (Randels-Thorp and Liss, 2016). Phosphorus levels are maintained through intestinal absorption and renal excretion (Barton and Kirby, 2017).
Calcium is an extracellular cation that is responsible for muscle contraction, blood coagulation, and various enzyme activities (Randels-Thorp and Liss, 2016). Most calcium contained in the body is in bone (99%) with the remaining 1% in the ECF and ICF spaces as ionised calcium (Barton and Kirby, 2017). Calcium is hormonally regulated, and levels are maintained by the GI tract and kidneys (Scalf, 2014).
Magnesium is an intracellular cation that is responsible for cellular energy metabolism, cell replication, protein synthesis, and is a catalyst for over 300 enzyme activities within the body (Randels-Thorp and Liss, 2016). Magnesium levels are maintained within narrow limits, however very little is known about the regulation mechanisms (Barton and Kirby, 2017).
The electrolyte status in critically ill patients influences the neurological, cardiovascular, muscular, renal, and GI body systems with clinical manifestations from the electrolyte abnormality/imbalance. Sodium disorders can cause life-threatening movement of water into or out of brain cells in response to osmotic shifts. Potassium disorders can cause life-threatening complications associated with ventilation, heart contractility and rhythm, and neuromuscular functions. Chloride disorders are closely linked to sodium transport and are related to changes in acid–base status. Phosphorus disorders can disrupt receptor-ion channels and membrane-associated ion pumps, ATP production, and regulation of cardiac output and vascular resistance. Calcium disorders can cause cardiac arrhythmias, renal dysfunction (i.e. tubular damage, mineralisation) and neurological dysfunction (i.e. seizures, neuromuscular excitability, skeletal muscle weakness). Magnesium disorders can affect normal neurotransmission, cardiac conduction and contractility, vascular tone, and skeletal muscle activity (Barton and Kirby, 2017).
Electrolytes are monitored through physical patient assessment and laboratory testing. Physical examinations should be repeated at a minimum of twice daily for early detection of clinical consequences of an electrolyte abnormality (Liss and Norkus, 2019). Point of care laboratory testing of an electrolyte panel should be performed daily or more frequently, depending on the severity of the electrolyte disorder (Barton and Kirby, 2017).
Acid–base refers to the regulation of the body's internal environment through pH (Scalf, 2014). Dysregulation of the body's acid–base status can affect the ability to synthesise and utilise proteins, maintain cellular integrity, transport intracellular and extracellular molecules, and maintain essential body functions to sustain life (Hopper, 2015). The body's level of acid or base is determined by measuring the amount of hydrogen ions within the blood. The pH scale goes from zero (most acidic) to 14 (most basic/alkaline), with normal pH being in the very narrow range of 7.35–7.45 (Liss and Norkus, 2019). Acidosis refers to a disease process in the body that decreases the pH, while alkalosis refers to a disease process in the body that increases pH. To help regulate pH, the body utilises a buffering system and physiologic responses (Scalf, 2014). There are three major pathways within the body to maintain pH within the normal range:
- Regulation of the partial pressure of carbon dioxide (PCO2) with changes in alveolar ventilation
- Buffer systems to regulate bicarbonate (HCO3) and other acids
- Modification of the rate of renal excretion of acids and bases (Hopper, 2015; Randels-Thorp and Liss, 2016).
There are four primary acid–base disturbances in critically ill animals: metabolic acidosis, metabolic alkalosis, respiratory acidosis, and respiratory alkalosis.
Metabolic acidosis is the most common acid–base disturbance in veterinary medicine and is characterised by a low pH, low HCO3, and compensatory low PCO2. Metabolic acidosis occurs due to an increase in the amount of acid in the body. The body responds to metabolic acidosis by implementing buffering systems (renal excretion of ammonia) and hyperventilation (to increase alveolar ventilation) in an effort to raise the pH. Primary causes that contribute to metabolic acidosis include a loss of bicarbonate-rich fluids (i.e. small intestinal diarrhoea), addition or production of an acid (i.e. toxin ingestion, lactic acidosis, diabetic ketoacidosis), or failure of renal excretion of an acid (i.e. hypoadrenocorticism, renal failure) (Scalf, 2014; Wheeler and Kovacic, 2017; Liss and Norkus, 2019).
Metabolic alkalosis is characterised by a high pH, high HCO3, and compensatory high PCO2. The body responds to metabolic alkalosis by converting buffers, increasing renal excretion of alkali, and hypoventilation (to decrease alveolar ventilation) in an effort to lower the pH. Primary causes that contribute to metabolic alkalosis include loss of chloride-rich fluid (i.e. vomiting), severe potassium or magnesium deficiency, hypoalbuminaemia, refeeding syndrome, impaired renal function, post-hypercapnia syndrome, pyloric obstruction, chronic administration of an alkali, or diuretic treatment (Scalf, 2014; Wheeler and Kovacic, 2017; Liss and Norkus, 2019).
Respiratory acidosis is characterised by low pH, high PCO2, and compensatory high HCO3. The body responds to respiratory acidosis by buffering and renal absorption of HCO3, in an effort to raise pH. Primary causes that contribute to respiratory acidosis include hypoventilation, gas exchange disorders (i.e. diffusion impairment, ventilation-perfusion mismatch, shunt), or increase in CO2 production (Scalf, 2014; Wheeler and Kovacic, 2017; Liss and Norkus, 2019).
Respiratory alkalosis is characterised by high pH, low PCO2, and compensatory low HCO3. The body responds to respiratory alkalosis by buffering and renal excretion of HCO3, in an effort to lower pH. Primary causes that contribute to respiratory alkalosis include hypotension, fever/heatinduced illness, systemic inflammatory response syndrome, sepsis, pulmonary thromboembolism, pulmonary parenchymal disease, or hyperventilation (Scalf, 2014; Wheeler and Kovacic, 2017; Liss and Norkus, 2019).
Monitoring of acid–base status gives insight into three physiologic processes: alveolar ventilation (venous); acid–base (venous or arterial); and oxygenation (arterial). Monitoring of a patient's acid–base status is primarily done through either venous or arterial blood gas analysis. The values needed for acid–base interpretation are pH, PCO2, and HCO3. The pH is a measurement of acidity or alkalinity of the blood (how many hydrogen molecules are present in the blood). An excess of hydrogen ions causes a decrease in pH (acidosis), while a shortage of hydrogen ions causes an increase in pH (alkalosis). The PCO2 is a measurement of the partial pressure of carbon dioxide in the blood. PCO2 is an indicator of the respiratory component of a blood gas analysis, and an excess of CO2 causes an acidosis, while a shortage of CO2 causes an alkalosis. The HCO3 is a measurement of bicarbonate in the body. Bi-carbonate is a major buffer and represents the metabolic component of blood gas analysis. Acid–base status (i.e. blood gas analysis) should be monitored in critically ill patients daily or more frequently depending on the severity of the underlying disease process that is causing the acid–base disturbance (Liss and Norkus, 2019).
Mentation
Mentation status, or level of consciousness is a component of an overall neurological assessment. Level of consciousness refers to a patient's arousability and responsiveness to stimuli in their environment and relates to the degree of neurological injury (Iacovetta, 2017). In critically ill patients, neurologic injury occurs in two phases: first primary injury occurs immediately from an insult/injury, it is finite and cannot be altered, reversed, or stopped; and secondary injury occurs following the initial insult (i.e. disease process of the insult/injury) from a combination of intracranial and systemic insults that result in a series of physiological consequences, it is preventable, treatable, and reversible (Scalf, 2014; Babyak and Backus, 2019).
During initial patient assessment, level of consciousness can be classified as normal (alert, responsive), dull/depressed/obtunded (slowed or inappropriate response to sensory stimuli) (Figure 2), stuporous (unconscious but rousable to noxious stimuli) (Figure 3), delirium (reduced state of consciousness with profound disorientation) or comatose (unconscious and unresponsive) (Scalf, 2014; Iacovetta, 2017). Changes in level of consciousness can be indicative of decreased cerebral function (i.e. lack of oxygen supply, change in cerebral perfusion), and should be addressed quickly to prevent further deterioration (Newfield, 2019).
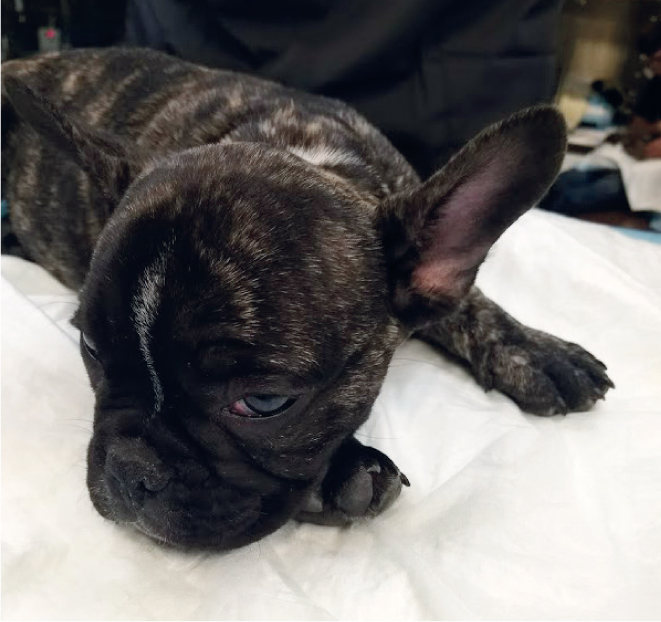
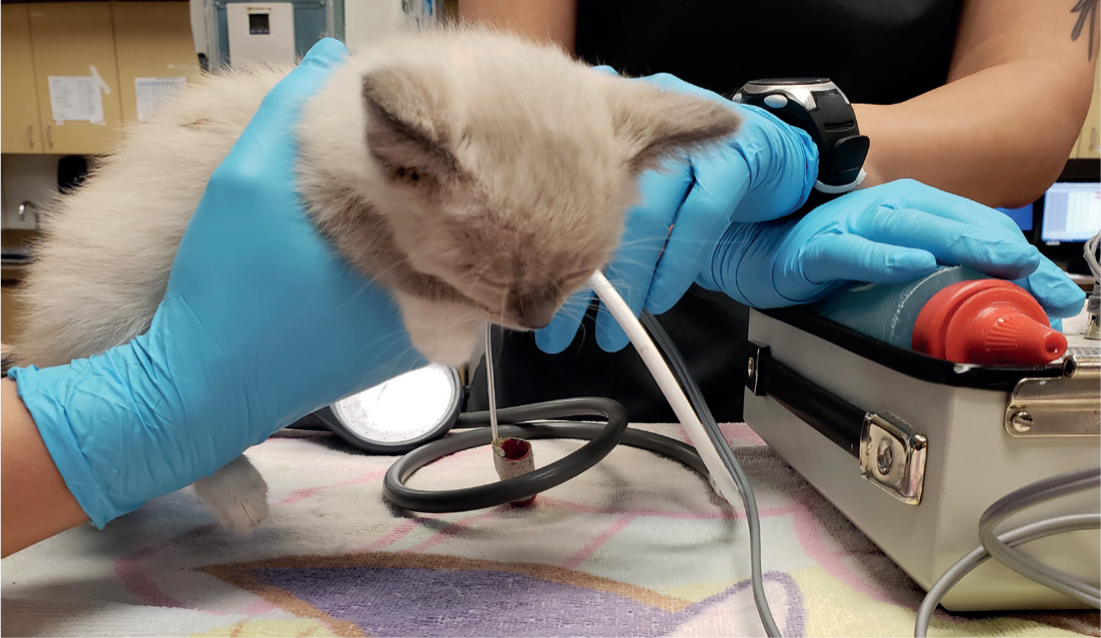
Level of consciousness is monitored using general patient observation and coma scales. One of the most used coma scales in veterinary medicine is the Modified Glasgow Coma Scale (MGCS) (Platt, 2015). The MGCS can be used to record progression or regression of the neurologic status over time. The MGCS involves three separate categories, including level of consciousness, motor activity, and brainstem reflexes (Table 1). This scale system is beneficial because it allows for an objective evaluation of the patient that can be used to monitor progression or deterioration of their neurological state, as well as has been shown to be a prognostic indicator (Figure 4) (Platt, 2015). Critically ill patients should have their mentation/level of consciousness serially monitored as part of routine patient parameters (i.e. every 1–4 hours), because patient neurologic status can change rapidly (Iacovetta, 2017).
Table 1. Summary of the Modified Glasgow Coma Scale (MGCS) used for neurological assessment in canine and feline patients. Each category has a score of 1 to 6 that represents the patient's clinical signs from mild to severe
Motor activity | Score |
---|---|
Normal gait, normal spinal reflexes | 6 |
Hemiparesis, tetraparesis or decerebrate activity | 5 |
Recumbent, intermittent extensor rigidity | 4 |
Recumbent, constant extensor rigidity | 3 |
Recumbent, constant extensor rigidity with opisthotonos | 2 |
Recumbent, hypotonia of muscles, depressed or absent spinal reflexes | 1 |
Brain stem reflexes | Score |
Normal pupillary light reflexes and oculocephalic reflexes | 6 |
Slow pupillary light reflexes and normal to reduced oculocephalic reflexes | 5 |
Bilateral unresponsive miosis with normal to reduced oculocephalic reflexes | 4 |
Pinpoint pupils with reduced to absent oculocephalic reflexes | 3 |
Unilateral, unresponsive mydriasis with reduced to absent oculocephalic reflexes | 2 |
Bilateral, unresponsive mydriasis with reduced to absent oculocephalic reflexes | 1 |
Level of consciousness | Score |
Occasional periods of alertness and responsive to environment | 6 |
Depression or delirium, capable of responding but response may be inappropriate | 5 |
Semi comatose, responsive to visual stimuli | 4 |
Semi comatose, responsive to auditory stimuli | 3 |
Semi comatose, responsive only to repeated noxious stimuli | 2 |
Comatose, unresponsive to repeated noxious stimuli | 1 |
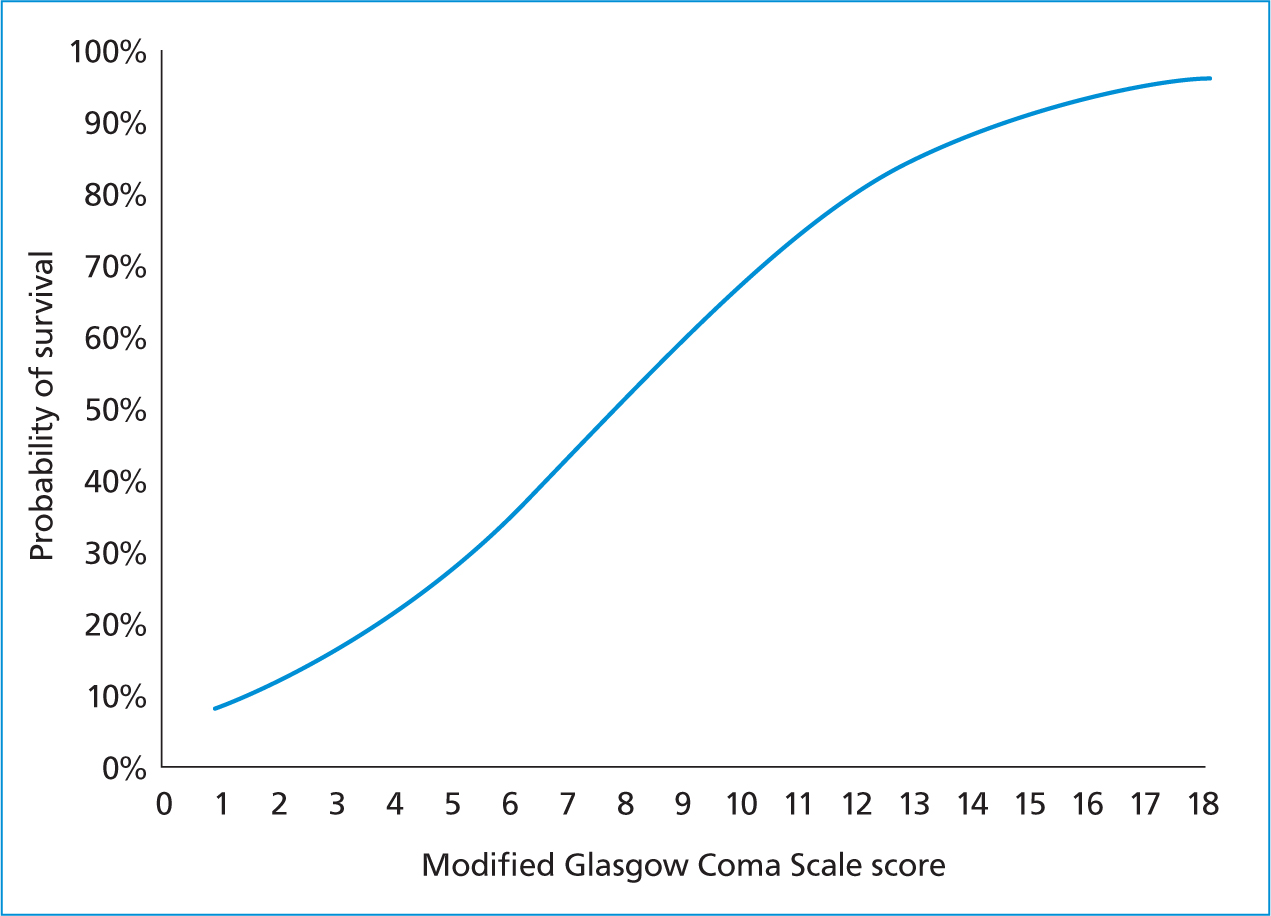
Heart rate, contractility, rhythm
The main function of the cardiovascular system is to transport oxygen to the rest of the body with the heart working as a pump to circulate oxygenated blood. When assessing cardiac function, consideration needs to be given to both the mechanical and electrical aspects.
The mechanical aspect of heart function is the pumping action of the heart to generate heart rate and contractility. Heart rate represents the number of heartbeats (cardiac cycles) in a minute and is a by-product of appropriate cardiac output (driving force for systemic blood flow) (Burkett, 2017). Contractility refers to the strength of heart contraction during each heartbeat (Scalf, 2014). Critical illness places significant demands on the cardiovascular system, and the heart must be capable of adjusting heart rate and contractile forces to meet the oxygen needs of the patient (Burkett, 2017).
The electrical aspect of heart function is the heart rhythm. Heart rhythm represents the electrical activity of the heart during the cardiac cycle (Schumacher, 2016). The cardiac cycle involves: P wave, atrial depolarisation; QRS complex, ventricular depolarisation; and T wave, ventricular repolarisation (Mellema and Kohen, 2012).
Monitoring of heart rate, contractility, and rhythm (cardiac output) involves assessment of patient parameters (perfusion parameters) and diagnostic instrumentation (electrocardiogram (ECG)).
On routine patient assessment, the six perfusion parameters used to determine cardiac performance (i.e. appropriate oxygen delivery to tissues) include mentation, heart rate, pulse rate, mucous membrane colour, capillary refill time, and extremity temperature (Darbo and Page, 2017). A patient's mentation can be altered because of decreased cerebral function from lack of cardiac oxygen supply to the brain (Babyak and Backus, 2019). The chest should be auscultated to determine the heart rate and determine if there are any irregularities detected (i.e. murmur, arrhythmia). The pulse quality should be palpated and taken in conjunction with heart rate auscultation, and refers to the difference between the systolic and diastolic arterial blood pressure, which is reflective of adequate stroke volume (SV) (amount of blood pumped by the heart each beat) (Farry and Norkus, 2019). A patient's pulse quality can be described as normal (normal SV), weak (decreased SV), bounding (increased SV), or absent (failure of appropriate SV) (Scalf, 2014; Babyak and Backus, 2019). Mucous membrane colour can be evaluated by looking at the patient's gums, and provides information about peripheral capillary perfusion. During circulatory problems, mucous membrane colour changes in response to changes in peripheral perfusion: light/pale pink mucous membrane colour is indicative of a vasoconstrictive disease process (hypovolaemia), while injected/red mucous membrane colour is indicative of a vasodilatory disease process (i.e. distributive shock states) (Burkett, 2017). Capillary refill time provides further information about peripheral perfusion and can be classified as normal, rapid (less than 1 second) or prolonged (greater than 2 seconds). Extremity temperature is evaluated by feeling the paws and distal limbs of a patient, and should normally be warm to the touch. Cool extremities indicate poor perfusion, as cardiac output diverts blood flow to the central circulation (Scalf, 2014).
ECG is a monitoring device that reflects the electrical impulses within the heart (Schumacher, 2016). Use of an ECG enables an evaluation of the heart's electrical components and is helpful in determining if an arrhythmia is present (Figure 5). Tachyarrhythmias and bradyarrhythmias are common ECG findings in the critically ill, and being familiar with a normal ECG rhythm is important to allow recognition of an abnormality.
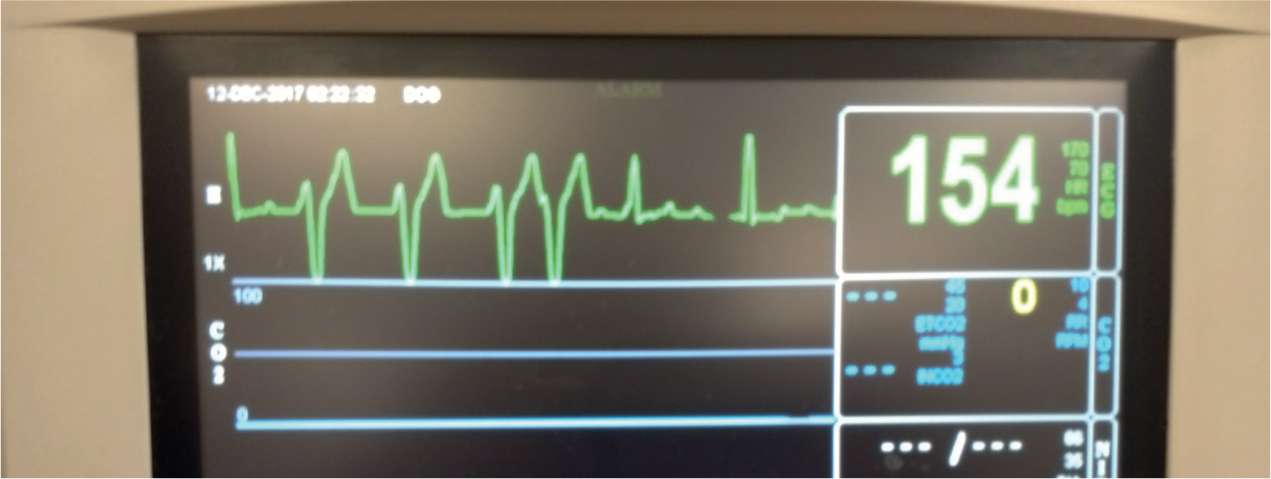
Conclusion
Veterinary nurses are the patient's primary caregivers and are involved in monitoring critical patients. Utilising the Kirby's Rule of 20 patient checklist provides an organised method for veterinary nurses to thoroughly assess the critical patient parameters of fluid balance, albumin and oncotic pull, electrolytes and acid–base, mentation, heart rate/rhythm/contractility. Having a thorough understanding of each of these patient parameters enables veterinary nurses to prioritise patient needs by assessing the overall clinical picture and elevating the standards for quality patient care.
KEY POINTS
- Kirby's Rule of 20 is an established, evidence-based patient checklist that can be used by veterinary nurses in the emergency and critical care setting to assess the overall clinical picture of critically ill patients, implement critical thinking skills, and elevate the quality of patient care.
- Fluid balance is a state of total body water homeostasis between the body's physiologic fluid compartments. Various disease conditions cause fluid imbalances, which require implementation of a fluid therapy plan and monitoring.
- Albumin is the predominant intravascular protein that is responsible for maintaining oncotic pull (force that holds water within the intravascular space). Hypoalbuminaemia is an anticipated complication of critical illness and necessitates monitoring.
- Electrolytes play a vital role in maintaining normal cellular functions. Acid–base is responsible for regulating the body's internal environment through pH. Electrolyte and acid–base disturbances can have clinical manifestations of major body systems and require monitoring.
- Mentation status, or level of consciousness, refers to a patient's arousability and responsiveness and is part of an overall neurological assessment. Changes in any of the five classifications of levels of consciousness can indicate decreased cerebral function, which warrants close monitoring.
- Heart rate, rhythm, and contractility are all components of the cardiovascular system that work to transport oxygen to the rest of the body. Heart function includes both a mechanical and electrical aspect that entails close monitoring.